Drawing Physics, From Aristotle’s Universe to Max von Laue’s X-Ray Crystallography
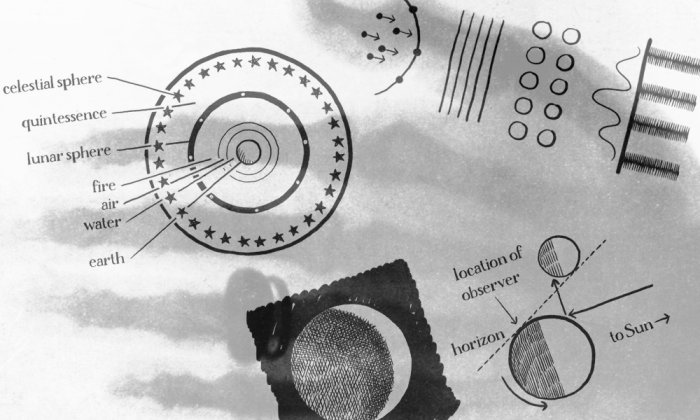
Humans have been trying to understand the physical universe since antiquity. Aristotle had one vision (the realm of the celestial spheres is perfect), and Einstein another (all motion is relativistic). More often than not, these different understandings begin with a simple drawing, a pre-mathematical picture of reality. Such drawings are a humble but effective tool of the physicist’s craft, part of the tradition of thinking, teaching, and learning passed down through the centuries.
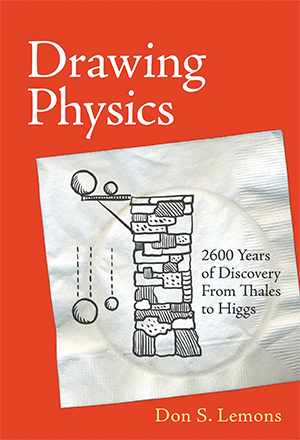
Don Lemons’ book “Drawing Physics” uses drawings to help explain 51 key ideas of physics accessibly and engagingly, broken up into sections covering periods from antiquity to the 20th century and beyond. Lemons, a professor of physics, pairs short, elegantly written essays with simple drawings that together convey important concepts from the history of physical science. We’re pleased to share a few of those texts, shining a light on Aristotle’s celestial spheres, Röntgen’s accidental x-rays (and von Laue’s subsequent diffraction by crystals), and Leonardo’s “earthshine,” the ghostly glow visible between the horns of a crescent moon.
—The Editors
Aristotle’s Universe (350 BCE)
Have you ever heard someone say “Eventually scientists will figure out how to do it?” You fill in the reference for “it.” Travel faster than the speed of light? Build a heat engine with 100 percent efficiency? Extract energy from the cosmic microwave background? Indeed, it may be that some things once thought impossible will turn out to be quite possible. But it is not true that everything of which we might dream is possible. After all, we live in a world that has a nature: a characteristic way of being and of becoming, of remaining the same and of changing.
We may learn about that nature and discover ways to employ it, but we have no power to change the nature of things. According to Francis Bacon (1561–1626), “Nature, to be commanded, must be obeyed.” Aristotle (384–321 BCE) transmitted to us this indispensable concept of nature — a concept rejected, perhaps unintentionally, by those who think scientists and engineers can do anything and everything.
The word nature comes to us from a Latin root, the Greek equivalent of which is φύσις or phusis, from which also derives physics. Of course, modern physics was born struggling against certain Aristotelian ideas. Nevertheless, Aristotle’s concept of nature is the bedrock upon which the practice of modern physics stands.
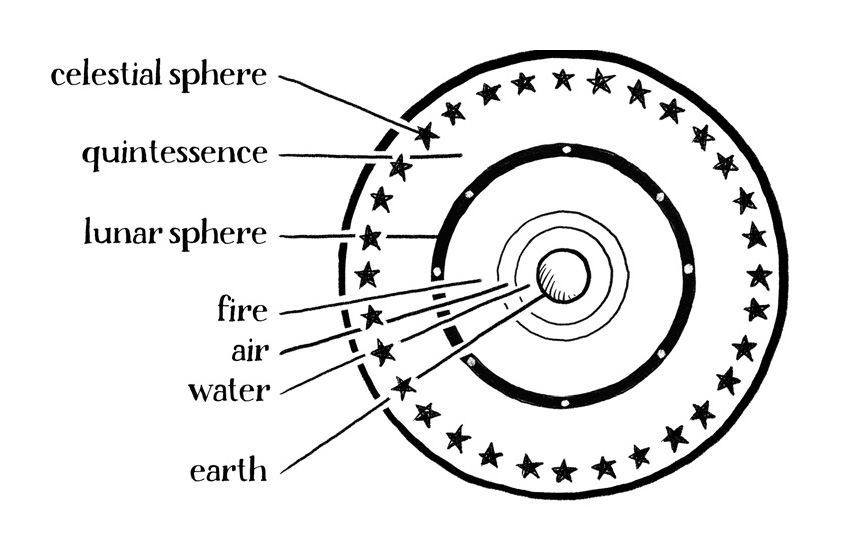
The drawing above illustrates Aristotle’s universe — not as one would observe it — but rather in the state of perfection toward which Aristotle’s universe tends by virtue of its nature. Earth and water move down toward the center — earth more persistently than water. Air and fire move up away from the center — fire more readily than air. Thus upward and downward motions characterize the region below the sphere of the moon. Objects above the lunar sphere are composed of a fifth substance, the quintessence or ether. The sun and wandering stars or planets (not shown in the diagram) and the fixed stars reside on transparent spheres that carry them around the earth in concentric circles. Circular motion characterizes the region above the sphere of the moon.
Aristotle borrowed many of the features of his universe from his pre-Socratic predecessors, for instance, the four elements (earth, air, fire, and water) and the celestial spheres. Furthermore, the pre-Socratics were the first to formulate the concept of nature. But Aristotle composed these ideas into an ordered whole, a cosmos, that answered the questions of his day and, at the same time, remained consistent with commonplace observations.
According to Aristotle, the realm of the celestial spheres is perfect. Its motions are completely natural, manifestly beautiful, and ultimately caused only by the desire for the good.
That last statement needs to be qualified for Aristotle must have observed sublunary objects that do not always travel up or down. Toss a clod of earth, and it travels along an approximately parabolic arc, at first up, then down, and always in the direction thrown. According to Aristotle, motion requires a mover and, if that mover is not the nature of the moving object, motion must be imparted and maintained externally, that is, unnaturally or by “violence.” Thus, it is the hand that throws the clod and the air through which the clod moves that cause its unnatural horizontal movement.
According to this view, to manipulate objects and study their behavior, that is, to perform experiments, is not a reliable way to study nature. For in doing so, one fruitlessly studies that which has no nature: the whimsy of the human boy, for instance, that tossed the clod in a particular way. To manipulate a natural phenomenon, is to spoil its naturalness — at least according to Aristotle.
Nevertheless, Aristotle was a great observer of nature and, according to the eminent historian of science George Sarton, “one of the greatest philosophers and scientists of all times.” He discovered the law of the lever and was the first to systematically study meteorology. He “carried on immense botanical, zoological, and anatomical investigations [and] clearly recognized the fundamental problems of biology: sex, heredity, nutrition, growth, and adaptation.” He structured the elements of logic and originated the inductive method. Aristotle also wrote ageless treatises on literary criticism, ethics, and metaphysics. Indeed, there is hardly a branch of human knowledge to which Aristotle did not contribute.
In 335 BCE Aristotle established a school of philosophy and science in Athens called the Lyceum. Those who studied with and followed Aristotle became known as peripatetics, that is, those who study while walking from place to place. Aristotle’s most famous pupil, Alexander the Great, the son of Phillip II of Macedon, conquered the known world.
According to Aristotle, the realm of the celestial spheres is perfect. Its motions, unlike those of the sublunary realm, are completely natural, manifestly beautiful, and ultimately caused only by the desire for the good. It is not hard to see why Aristotle’s view of the universe has influenced thought and literature for over 2,000 years. It is, after all, a privilege and delight to look at the heavens each night and be inspired by their perfection.
Leonardo and Earthshine (1510)
If “Drawing Physics” had a section called “Renaissance Science,” Leonardo da Vinci (1452–1519) would be its exemplar. Yet, while Leonardo was of the Renaissance, he was not the kind of scholar glorified by the humanists of his time: a scholar nurtured in classical history and literature, having perfect Latin, skilled at rhetoric, and able to speak with confidence at public gatherings. Rather, Leonardo’s education was incomplete, his Latin was poor, and he had little interest in public affairs. But Leonardo was a keen observer of nature, an avid experimentalist, and a man drawn to practical applications. While the classically educated scholars of the Italian Renaissance quoted authors, Leonardo cited experience.
Leonardo poured much of his experience into 13,000 notebook pages of drawings and text — pages that have enriched the language of visualization. He originated the aerial view so helpful in topography and mapmaking and the idea of presenting different sides of the same object, for instance, of the aorta of an ox. He pioneered the use of anatomical cross-sections, and observed that, at the same distance, a bright object appears larger than a less bright object of the same size.
Leonardo may have intended his notebooks to comprise a profusely illustrated encyclopedia of all technical knowledge. But, as they come to us, their pages have no order other than that imposed by the vicissitudes of Leonardo’s life. He usually wrote from right to left with characters slanting leftward: so-called mirror-image cursive. We do not know whether this practice was meant to preserve the privacy of his entries or was simply more convenient for the left-handed Leonardo.
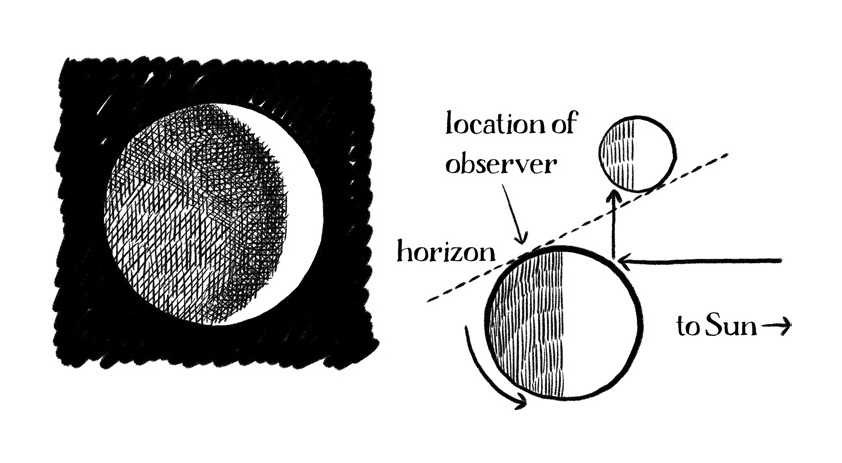
The notebooks do, however, help us understand how Leonardo could be a prolifically inventive genius and yet have so little influence on the development of science. Like Archimedes, he focused on isolated problems. But, unlike Archimedes, Leonardo failed to develop collections of coherent ideas that explain more than the subject at hand. It is as if the very fertility of his mind and the concreteness of his artistic vision fragmented his scientific efforts and, in this way, kept him from developing powerful, abstract theoretical explanations. Even so, the fragments of his thought are often intriguing. The drawing above illustrates one of them, on earthshine.
When the moon is a waxing or waning crescent, the shaded, relatively dark surface between the horns of its crescent glows with a faint, ghostly light — as suggested in the left panel of the drawing. Leonardo’s explanation of earthshine, illustrated in the right panel, is the earliest documented explanation of this phenomenon. According to Leonardo, a significant part of the sunlight striking the earth is reflected from its surface. The fraction of sunlight that reflects from the earth’s surface, known as its albedo, is close to 30 percent. Some of this reflected light strikes the dark side of the moon and some of that light is reflected back to the earth and observed as earthshine.
Leonardo got one detail of his explanation wrong. He believed that sunlight reflects primarily from the earth’s oceans, in particular from the tops of ocean waves. In fact, the earth’s clouds reflect much more sunlight than do its oceans. Photos taken from orbiting spacecraft confirm that the brightest parts of the earth are its cloud-covered areas. And when the earth’s cloud cover changes, the albedo of the earth also changes. In contrast, the moon has virtually no atmosphere and its albedo, about 12 percent, remains constant in time. Therefore, measuring changes in the intensity of the earthshine is equivalent to measuring changes in the earth’s albedo. The latter has become an important input to climate change models.
When walking the streets of Florence and Milan, Leonardo carried a notebook in which he sketched whatever caught his attention: people, buildings, and landscapes. On occasion, he would follow a stranger for hours until he could rough out their visage on paper. Leonardo also drew what he could only imagine: flying machines, cannons that shot exploding shells, and shoes that allowed one to walk on water. He designed a car powered by two sets of springs. While one set unwound and propelled the car forward, the car’s passenger would wind the other set. He envisioned a rotating spit powered by the same fire that cooked the flesh impaled upon it and a house of prostitution with an unusually large number of doors. Many of his designs are of practical devices that would, in time, be built. But no one has yet constructed Leonardo’s wake-up device contrived of mechanical relays that, when triggered by a water clock, jerked a sleeper’s feet into the air.
Leonardo was also keenly interested in mathematics and prepared illustrations for a mathematical text “De Divina Proportione”(1509) written by his friend Luca Pacioli. But, of course, Leonardo is most famous for his paintings — above all, “The Last Supper” and “The Mona Lisa” — paintings with animated postures; expressive faces; modeled, pointing hands; and pyramidal compositions. Leonardo painted in oils with a broader range of light and dark shades than is usually seen with the eye — a technique art historians call chiaroscuro. It may have been that the artist in Leonardo was drawn to the chiaroscuro of earthshine while the scientist in him sought its explanation.
X-rays and Crystals (1912)
On November 8, 1895, while experimenting with a beam of electrons created within an evacuated glass tube, Wilhelm Conrad Röntgen (1845–1923) accidentally discovered certain “rays” that propagated beyond the end of his tube. These rays seemed to travel in straight lines, made florescent materials glow, and exposed photographic plates. Because the rays traveled through flesh but not through bone, Röntgen used them to photograph the bones in his wife’s hand. He called them X-rays. Röntgen’s X-rays were immediately hailed as a new method of photography. The New York Times covered Röntgen’s discovery early in 1896. That year more than 1,000 professional and popular articles and 50 books and pamphlets were published on X-rays. Röntgen, however, was not pleased with the publicity and complained, “I could not recognize my ownwork in the reports.” But he had started something new. That spring the young Ernest Rutherford wrote to his fiancée that “every Professor in Europe is now on the warpath” trying to understand X-rays.
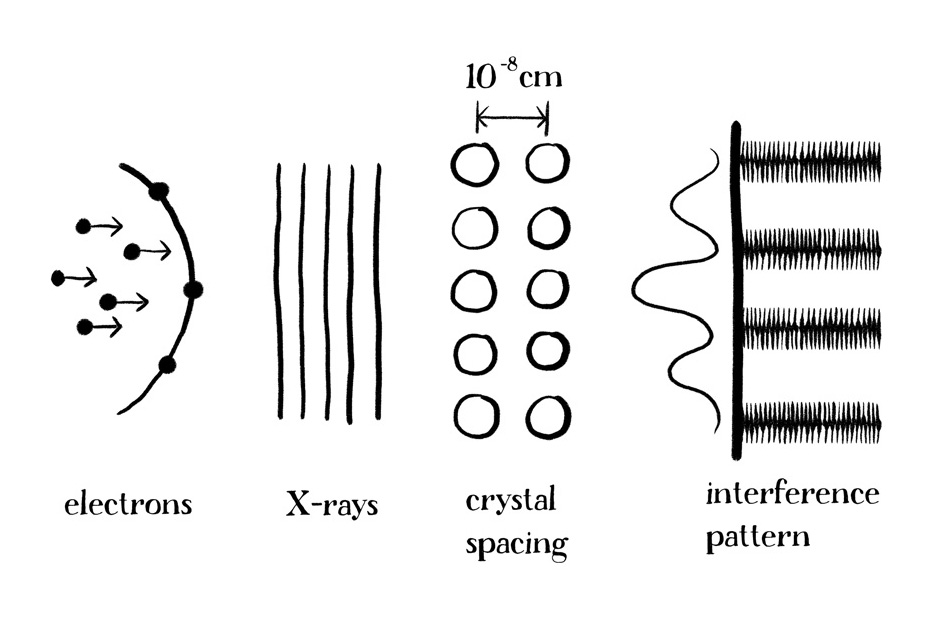
That understanding came slowly, but by 1912 evidence was accumulating that X-rays were very high frequency, short-wavelength electromagnetic waves. The left half of the illustration above depicts this understanding. Electrons are accelerated to high speeds and collide with the end of an evacuated glass tube. In the collision short-wavelength, electromagnetic waves — the X-rays — are created that carry forward the energy and momentum of the electrons. Yet not everyone was convinced. Some continued to believe that X-rays were particles.
Max von Laue (1879–1960), a near contemporary and friend of Albert Einstein, proposed an experiment (shown in the right half of the diagram above) whose result secured the case for waves. Early in 1912, while listening to a student explain his research on the interaction of long-wavelength electromagnetic waves with the atoms or molecules that compose a crystal, von Laue asked himself, “Why not shine X-rays on a crystal?” Since the spacing between the atoms or molecules in a typical crystal (10-8 cm) is only a little larger than the estimated wavelength of X-rays (10-9 cm), X-ray waves should, after passing through the crystal, produce an interference pattern, that is, a pattern of constructively and destructively superposing waves. This interference pattern should be similar to that produced by visible light passing through a regular series of parallel slit-shaped openings called a diffraction grating. In both cases, the interference pattern produced depends upon a wave property called diffraction, that is, a departure from straight-line propagation.
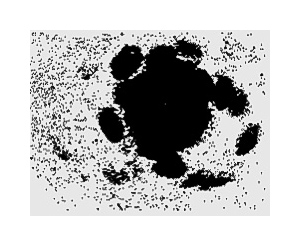
Although X-ray interference is, in its geometry, a smaller-scale version of visible light interference, physically the two cases are quite different. X-rays pass through a crystal by vibrating the charged particles in the atoms (or molecules) composing the crystal. These atoms, in turn, radiate new waves that pass on the interaction from atom to atom until the very last atoms on the far side of the crystal radiate like a string of regularly spaced radio beacons. Visible light, on the other hand, passes freely though the slits of a diffraction grating and is absorbed or reflected by the material surrounding the slits. Von Laue convinced two colleagues, Walter Friedrich and Paul Knipping, to test his idea. Their initial experiment, with materials and equipment on hand, captured on film the X-ray interference pattern shown to the right. It consists of several dark splotches, each indicating the constructive interference of diffracted X-rays, surrounding a single, larger dark patch, indicating the remains of the original ray. This image attracted favorable attention and secured funding for more refined experiments that fully confirmed von Laue’s detailed analysis. Von Laue, Friedrich, and Knipping published their first results in June 1912.
Von Laue’s rise, from junior faculty to Nobel laureate, took less than three years.
Von Laue’s idea was brilliant and its confirmation complete. In place of pursing a single idea for many years, he had “suddenly … perceived the way which subsequently proved to be the shortest path to success.” The Nobel committee awarded Von Laue its 1914 Prize in Physics for “his discovery of the diffraction of X-rays by crystals.” Von Laue’s rise, from privatdozent (junior faculty with no regular salary) to Nobel laureate, took less than three years.
Von Laue survived long enough to be tested by the fires of Nazism and World War II. He spoke out publicly against the persecution of the Jews and the promotion of a “German science” that, for instance, rejected relativity because Einstein was Jewish. He remained in Germany during the war, an outspoken critic of the Nazis, secretly helping his Jewish colleagues emigrate and then escape. After the war von Laue helped rebuild Germany’s institutions of science. Then, in 1960, a motorcycle struck and overturned the car he was driving to work. In the few days left to him, von Laue composed his own epitaph: “He died trusting in God’s mercy.”
Don S. Lemons is Professor of Physics Emeritus at Bethel College in North Newton, Kansas and the author of several books, including “Thermodynamic Weirdness,” and “Drawing Physics: 2,600 Years of Discovery from Thales to Higgs,” from which this article is excerpted.