The Organ-on-a-Chip Revolution Is Here
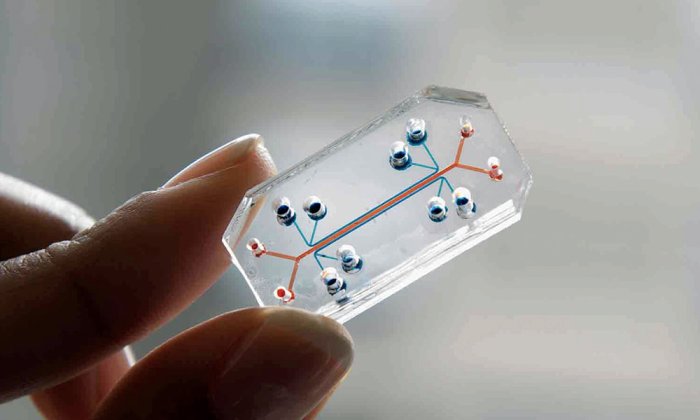
Jim Grotberg grew up using his lungs a lot, and later became professionally allured to them. He played trombone in high school and went on to play basketball with Cornell Big Red, Cornell University’s NCAA Division 1 men’s basketball team. When he became a doctor-researcher and a world expert in biofluid dynamics, his fascination with the crackling sounds he heard while listening to the chests of patients with a stethoscope stayed with him.
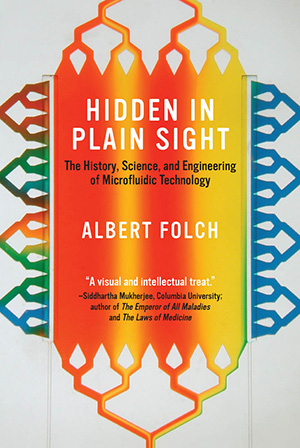
In the late 1990s, Grotberg was studying airway narrowing, a phenomenon that occurs when the lung’s airways become clogged with fluid. In a healthy lung, the airways are usually lined by a thin liquid film that keeps the tissue moist. Under certain conditions — when a virus causes inflammation, for example — the tissue reacts by producing much more fluid, which causes the airways to narrow. When that happens, there’s a higher chance of the passage being blocked by a liquid plug that moves down the airways until it ruptures. With a stethoscope, doctors can hear the plugs rupturing (that crackling sound) and infer how your lungs are doing. Grotberg was trying to prove his hypothesis that the liquid plugs causing the crackling sounds were harming the cells lining the airways — but this was difficult to do without building a system that could properly observe the lung’s airways, with cells and all. And then it occurred to him: Maybe he could use lung cells in microfluidic channels to study the sound.
Most people know what microelectronics are: those small but mighty components powering our phones, TVs, and other electronic devices. Microfluidics is a related miniaturization technology but applied to fluids instead of electricity. Often hidden from view, microfluidics underlies a variety of devices that are essential to our lives, from home pregnancy tests to inkjet printers to glucometers for the monitoring of diabetes. In recent years a class of microfluidic devices, called organs-on-chips, have even been used to mimic the natural environments of organs, opening the door to experiments that would otherwise not be feasible. This, it turned out, was exactly the technology Grotberg was looking for.
Often hidden from view, microfluidics underlies a variety of devices that are essential to our lives, from home pregnancy tests to inkjet printers to glucometers for the monitoring of diabetes.
By a stroke of luck, in 1999, Shuichi Takayama, a bright chemist-turned-microfluidic engineer who was finishing up his postdoc at Harvard, interviewed for a faculty position at Grotberg’s department at the University of Michigan. At Harvard, Takayama had shown that cells could be kept alive inside a microfluidic channel made of a transparent, oxygen-permeable rubber. In his seminar, Takayama lectured about vascular endothelial cells — cells that line the vessels of the circulatory system — and microfluidics. “It occurred to me that I could get him involved in airway cells and plug flows using cell-lined channels,” Grotberg recalls. “So we hired him and I shared my student Dan Huh to develop the interaction.”
In 2004, Takayama and Huh started ruminating on the design of a microfluidic device that could mimic the biomechanical functions of the lung. Since we can’t just insert a microscope into the lung, little is known about how infection occurs at the cellular and tissue level and the effect of important physiological parameters such as the speed of inhalation. Takayama and Huh wanted to gain insight into how smoke damages lungs, how viruses infect them, and whether new vaccines could work.
The first step was to see if they could culture primary airway cells in a microfluidic device and keep them alive. Airway cells, as the name suggests, are the cells that are in contact with air inside the lungs. Fortunately, human airway cells were commercially available.
For the first lung-on-chip design, in 2005, Huh found inspiration in Transwell inserts, a simple device that consists of a porous membrane where cells are seeded. Researchers had previously shown that airway cells could stay alive grown on the porous membrane of the inserts while exposed to air from the top and fed with nutrients dissolved in cell culture medium from below. To imitate the Transwell structure, he put a PDMS channel — essentially a transparent rubber mold — on top, another one at the bottom, and sandwiched a porous membrane in the middle. “That design was key to differentiating cells in a device because we had to culture them under air while feeding them from the bottom,” says Huh. “It worked beautifully,” he remembers.
The next critical question was whether plugs could be generated in a way that mimicked the movement of actual fluid plugs, and whether they would imitate the crackling. Here, too, they were successful. Better yet, when the plugs ruptured, cells would die, “so we were able to claim that crackling might be associated with mechanical lung injury,” Huh adds. Their paper was published in 2007 in PNAS. It was a landmark moment, the first of many.
Not long after, Huh was invited to continue his work at Don Ingber’s lab at Harvard. Ingber had heard a lecture by Takayama around 2005 presenting the microfabricated liquid plug generator. “I was amazed by [Takayama’s] presentation because the plugs moving through the channels generated a sound that was exactly the same as the ‘crackle’ sound I was taught to listen for through a stethoscope during a lung exam when I was a medical student,” says Ingber. “This truly was amazing to me because every med student in the world would ask their professor what caused that sound, and no professor could ever provide a good answer beyond ‘fluid in the lungs,’” he adds. During Huh’s postdoc interview in 2006, Ingber told Huh that “what would be really exciting would be to build a real living breathing lung lined by living cells with human tissue–tissue interfaces that could be visualized and studied in vitro.”
“This truly was amazing to me because every med student in the world would ask their professor what caused that sound, and no professor could ever provide a good answer beyond ‘fluid in the lungs.'”
Upon this suggestion, Huh started the arduous task of building a model of the alveoli, the small sacs at the tip of the airways where gas exchange between air and blood capillaries occurs. “That’s where a lot of important things happen,” insists Huh. “I tried really hard to design some kind of a mechanical stretcher that could be integrated into a microfluidic device where I could culture alveolar cells and then stretch and relax them to mimic breathing motions,” he recalls. Finally, while dozing off at church, the inspiration for a critical feature of the device — a flexible flap that allowed him to pneumatically inflate the device and replicate lung inhalation — came to him.
Huh presented the design at a group meeting, where it received general praise from the senior scientists. In a critical demonstration, Ingber and Huh placed bacterial cells on the channel that contained lung cells, then they introduced white blood cells into the channel that contained the endothelial cells, and showed the entire process of immune cell adhesion, diapedesis (the movement of white blood cells through capillary walls), and phagocytosis (the engulfing of bacteria by a white blood cell) in the lung compartment. In other words, they mimicked complex responses that only happen at the organ level — on a chip. Their results were published in Science.
Using the same lung-on-a-chip device, Huh next modeled pulmonary edema (sometimes called “wet lung”) processes induced by drug toxicity observed in human cancer patients treated with interleukin-2 (IL-2), a type of signaling protein that regulates the activities of white blood cells. The chip allowed for reproducing mechanical forces associated with physiological breathing motions. The study revealed that these motions play a crucial role in the development of increased vascular leakage leading to the condition. Importantly, and without performing a single animal test, this work led to identifying potential new drugs that could prevent IL-2 toxicity in the future.
Eventually their systems caught the attention of NASA, who wanted to learn why half of the astronauts who flew in Apollo missions reported minor bacterial or viral infections within a week of their return, and who sought to “better understand the role of microgravity on human health and disease and to translate that understanding to improved human health on Earth.” The agency recently launched a “Tissue Chips in Space” initiative.
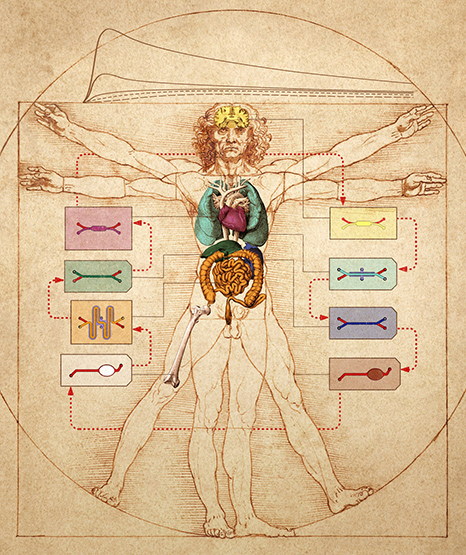
Other researchers quickly realized the organ-on-a-chip concept could be extended well beyond the lung. “We started getting inquiries and calls from pharma right away, and Don [Ingber] started a sponsored research agreement with Merck,” remembers Huh proudly. Don Ingber launched the Wyss Institute at Harvard in 2009 with a broader mission to develop biomimetic technologies such as organ-on-a-chip and 3D printing. After the lung-on-a-chip, Ingber had the idea of developing a gut-on-a-chip device as an extension of the lung-on-a-chip concept. During Dan Huh’s second year as a postdoc in Ingber’s group, Hyun Jung Kim joined the lab with a background in microbiology. Kim developed the first gut-on-a-chip mimicking the peristaltic (waves of squeezing) function of the gut, a paper that raised much interest.
The Wyss Institute has since generated a total of 15 microphysiological models of living human organs, including the lung, intestine, kidney, skin, bone marrow, and blood-brain barrier, among others. To mimic the physiology of the whole human body and predict tissue responses to drugs, Wyss researchers have developed an instrument that links up to 10 different organs-on-chips by transferring fluid between their common vascular channels. As a testament to its success, Wyss now employs about 300 staff and has become the flagship for organ-on-a-chip research and biologically inspired engineering in the world. “It truly ‘takes a village’ to build something big,” emphasizes Ingber.
Researchers around the world have followed suit, addressing a host of challenges: Abe Stroock’s lab at Cornell developed a vascularized device made only with biological components, an achievement that seemed impossible only a decade ago; a team led by Hans Clevers and Matthias Lutolf formed homeostatic miniature intestines, inside which gut stem cells grew and regenerated, allowing their group to model bacteria interactions essential to our gut well-being; a Dutch team led by Geny Groothuis and Elisabeth Verpoorte engineered a way to test in a device drugs on liver slices (perhaps one of the most challenging tissues to engineer due to its 3D and biochemical complexity, connectivity with other organs, and the difficulty of keeping it in its differentiated state); and, in 2016, Marco Rasponi’s group designed a “beating-heart-on-chip.” The list goes on.
One day in early 2000, as I was having lunch with biomedical engineer Mehmet Toner in Boston, a number of animal-rights protestors paraded down nearby streets. Inevitably, our conversation drifted to the relevance of animal research for human medicine. “If we researchers had always been forbidden from using animals for research, by now we would have found a way to answer all these questions directly in humans,” Toner reflected. The thought that we might be using animals out of convenience rather than out of necessity has stuck with me ever since. About a decade later, the elegance of the Wyss Institute organ-on-a-chip designs inspired a new line of inquiry in several labs, including mine, focused on cancer. Would it be possible to use microfluidics to test multiple cancer drugs on a small, live tumor biopsy? That would be a more efficient, direct-in-human test for drugs and would avoid unnecessary animal suffering.
Not to mention the potential for cost-savings. If you have been severely sick, you will have felt the unfairness of the high cost of drugs. The latest drugs for cancer, cardiovascular or gastrointestinal diseases, nervous disorders, and rare conditions are so costly that even selling an expensive car won’t cover a year’s supply of them.
The most poignant example is that of cancer drugs, whose approval is by far the most wasteful process of all drug types: The FDA approves less than four percent of cancer drugs, meaning 96 percent of them spend more than a decade being tested in petri dishes, mice, and a small set of patients, before scientists finally realize that they aren’t suitable for human use. Each drug in the four percent that does get approved bears an average price tag of more than a billion dollars, a bill passed down to you, the patient-customer.
Given what is at stake, it is not surprising that a growing number of companies are offering their organs-on-chips to pharma, each with a different technology, patented chip design, or organ. The three oldest companies in this space — Hurel Corporation, Hepregen, and Organovo, founded in 2007 — are all from the United States. InSphero (Switzerland, 2009), TissUse (Germany, 2010), and Mimetas (Netherlands, 2011) were started shortly afterward. Nortis (Seattle, 2012) and Emulate (Boston, 2013, by Don Ingber) followed, and after them half a dozen startup companies are fighting to grow in this exciting cauldron. Thanks to the organs-on-chips pioneered by Grotberg, Huh, and Takayama, the day when most new drugs will be developed and tested directly (and only) using human tissues is fast approaching.
Albert Folch is a leading researcher in microfluidics. He is Professor in the Department of Bioengineering at the University of Washington and the author of several books, including “Hidden in Plain Sight: The History, Science, and Engineering of Microfluidic Technology,” from which this article is adapted.