Calling Pandora: Bohdan Paczyński, Microlensing, and the Hunt for Exomoons
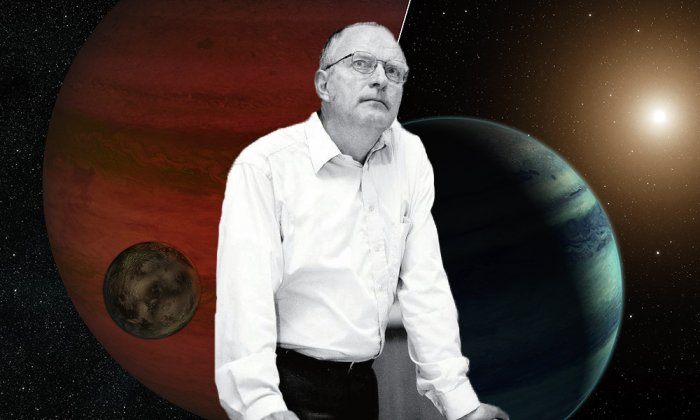
In James Cameron’s 2009 sci-fi epic “Avatar,” Pandora is one of many moons of the gas giant Polyphemus, named after the man-eating cyclops described in Homer’s “Odyssey.” Pandora is named after the first human woman in Greek mythology, who opened a jar and released the evils of humanity. (Inevitably, a stigma has been attached to Pandora over the centuries. In fact, Zeus did not punish her because he knew she would open the jar, and curiosity is a virtue in a scientist. She managed to close the jar and contain one evil before it escaped: hopelessness. As a result, humans have hope, and that has tided us through bad times.)
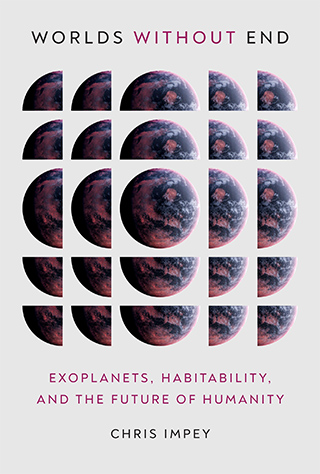
There’s a real moon called Pandora; it’s the 13th-largest moon of Saturn, small enough to fit inside London or Los Angeles. The real Pandora is airless, pockmarked by craters, and not nearly as interesting as Cameron’s fictional world. The Pandora in “Avatar” is slightly smaller and has weaker gravity than Earth. Mountains hang in midair buoyed on strong magnetic fields, created by an abundant superconducting mineral dubbed unobtanium. Its biosphere brims with bioluminescent diversity. The atmosphere has enough oxygen but too much carbon dioxide for humans to breathe, so the blue-skinned Na’vi have special organs to metabolize it.
Fanciful as Cameron’s Pandora may be, there’s good reason to believe that many, if not most, exoplanets may have moons in orbit around them. In the search for habitable worlds far out in space, it might therefore be worth looking at not only planets but also moons.
Earth is the only planet in our solar system within a traditionally defined habitable zone: The range of distances from the Sun where liquid water can exist on the surface of a planet. But if we assume that the only essential ingredients for biology are water, a local source of energy, and carbon-rich material, then life might exist in other locations in the outer solar system — and not only planets. Mars is too cold for surface water, but there’s convincing radar evidence for subsurface aquifers. The oceans of Jupiter’s moon Europa are also a good prospect, along with Saturn’s small moon Enceladus. Altogether there are a dozen moons in the cold depths of space where biology is conceivable, and the list even includes the dwarf planet Pluto, which is on average 40 times as far from the Sun as Earth is (40 astronomical units, or AU). Even this far from the Sun, water under a moon’s surface can be kept from freezing by a combination of pressure from overlying rock, radioactive heating within the moon’s rock, and tidal squeezing by the planet it orbits. Moons boost our solar system’s potentially habitable real estate substantially, and there is no reason to expect that it would be any different in other planetary systems. Yet detecting exomoons has proven to be quite the challenge; indeed, the first confirmed exomoon would be a big prize.
Altogether there are a dozen moons in the cold depths of space where biology is conceivable.
So far, the story sounds familiar from the early years of exoplanets. Claims retracted. Discoveries not confirmed by other groups. Detections on the hairy edge of the noise. Most searches have delved deep into data from the Kepler satellite. Exomoons are so small that only an enormous one could make a detectable blip in the light trace of a star, but the way they tug on the exoplanet they orbit affects the timing of eclipses. Astronomers at the University of Western Ontario used this “transit timing variation” to identify eight possible exomoons, but skeptical reanalysis left only two as viable candidates. Pulling such tiny signals from noisy Kepler data is extremely difficult. To be sure they’re not being fooled, astronomers ran simulations of billions of possible star-planet-moon combinations and compared them to actual Kepler data to look for a good match. This approach consumed five million hours of processing time on a powerful NASA supercomputer.
It turns out there’s another tool for finding exoplanets, and it’s also relevant in the search for exomoons. This cunning method relies on a central prediction of Einstein’s general theory of relativity: Mass bends light. Einstein replaced Newton’s concept of space and time as linear, independent entities with a theory that merged them into one entity, called space-time. His theory of gravity was geometric, and it emerged from the mathematics that mass could bend space-time.
In an analogy with optics, mass can bend, focus, and magnify light just like a lens can. The effect is called gravitational lensing. It’s most familiar from beautiful Hubble Space Telescope images of distorted and magnified galaxies. A foreground galaxy or cluster acts as the lens, and it magnifies and brightens the light of the background galaxies. If the alignment is right, a massive foreground galaxy bends light from the background galaxies to such an extent that we see multiple, distorted images of the background galaxies. A far less massive object like a star doesn’t bend light much — the angle is a tiny fraction of an arc second. But the light is still magnified and brightened. That’s called microlensing.
Our story of how it was used to detect exoplanets — and is the sharpest tool in the search for exomoons — starts with a 14-year-old Polish boy, brimming with intelligence. He grew up in grim, postwar Warsaw, a city obliterated by the Nazis and subjected to Soviet leader Joseph Stalin’s deadly purges. His name is Bodhan Paczyński. Young Paczyński was up way past his bedtime, observing an eclipsing binary star with a small telescope belonging to the Warsaw Observatory. The observatory, situated on the outskirts of town, was an abandoned property with half-destroyed buildings. Paczyński got there on the tram and then by walking four kilometers through a pine forest. There was no electricity, so he worked by candlelight. The data he took would become part of his first research paper, which was published when he was only 18. So began a lifelong infatuation with stars that whirl around each other in a dance where one crosses the face of the other.
Paczyński’s brilliance was recognized early on. His teachers helped him cross the Iron Curtain to do research. At age 22, he spent a year at Lick Observatory in California — the first of many visits to the West. He was part of a succession of young astronomers from Poland who worked at Lick. These immigrants were popular because they were not only good but also cheap since Poland was in dire economic straits. Each fresh recruit was trained by their predecessor in the tricks of the trade: how to smash dry ice and pack a cold box, how to get data off punch tape, and how to survive weeks alone on a mountaintop observatory. Locals were perplexed by Paczyński’s rapid, almost unintelligible English; he had learned the language by reading the highly technical Astrophysical Journal. Returning to Poland, he endured food shortages, married his childhood sweetheart, and got his PhD, finishing the calculations on a primitive vacuum tube computer.
Paczyński was insightful and voracious in his astronomical interests. In the 1970s, he solved five fundamental problems in stellar evolution. He wrote a prescient paper that predicted how binary star systems could be used to detect gravitational waves. At 36, he became the youngest-ever member of the Polish Academy of Sciences. He traveled abroad frequently since it was the only way he could get access to computers fast enough to do the calculations he needed for his work. Often, his family was essentially held hostage to ensure his return to Poland. In 1981, he was giving lectures at Caltech when martial law was declared in Poland. The regime started arresting and detaining intellectuals. Since his wife was with him on that trip, Paczyński decided to stay in the United States. He spent the rest of his career as a professor at Princeton University.
In the early 1980s, Paczyński recognized that the increasing size of CCD cameras, along with rapid advances in the computer processing of images, would allow searches for the rare situation where a star is lensed by an intervening star or planet. He coined the term “microlensing.” Most astronomers doubted the effect would ever be seen. Undaunted, he set up a collaboration and network of small telescopes to monitor the brightness of millions of stars night after night. In 2003, two decades after Paczyński first conceived of the project, microlensing delivered its first exoplanet.
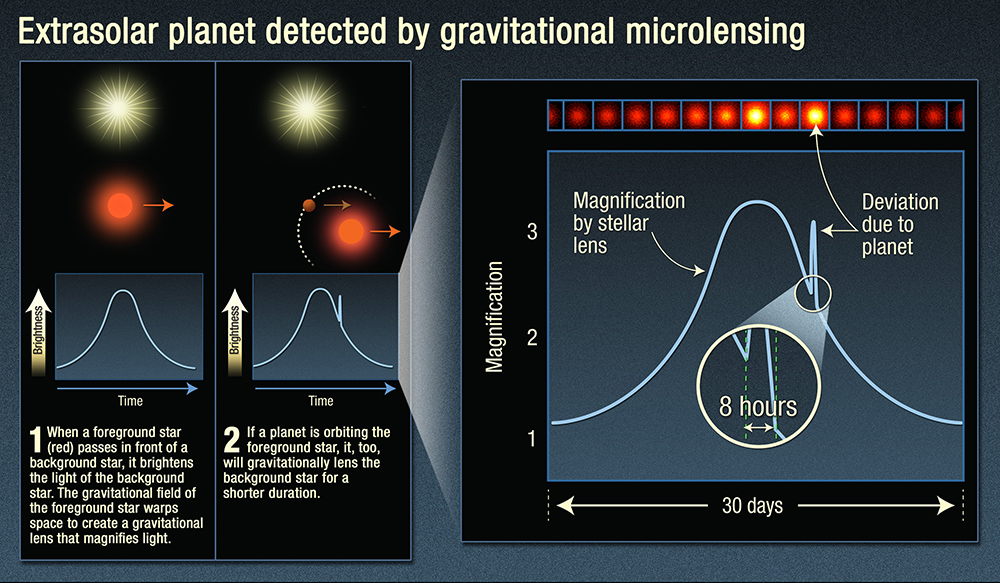
Here’s how it works. If one star is precisely in front of another star, the foreground star will magnify the light of the background star. It is a substantial effect; the brightening can be a factor of two or three. But stars are moving through space, so the perfect alignment is fortuitous and temporary. The brightening lasts a few weeks and then it fades over a few weeks, back to the normal level. Smaller and less massive objects magnify light by less and for a shorter time. For example, a planet five times Earth’s mass would brighten a background star by 10 percent for about eight hours. The effects of the planet (and any moon, if present) and star combine. This is another needle-in-a-haystack experiment. The odds of a perfect alignment are tiny, so hundreds of thousands of stars must be watched to catch one rare event. When the slow, monthlong brightening caused by the foreground star begins, astronomers observe the star frequently to try to catch the much-briefer brightening due to a planet. An orbiting exomoon would be an even smaller light blip on top of the slower magnifications, lasting just minutes. The moon is like a twig on a branch on a log in a vast forest.
As a graduate student, I had my own experience with looking for a needle in a haystack. My thesis work involved trying to find a rare type of active galaxy whose light output changed as the supermassive black hole that fueled the activity consumed nearby gas and stars. The active region is so small that the object looks like a faint star in the sky. In Australia, at the UK Schmidt Telescope, I took a series of photographic plates over weeks and months. There were hundreds of thousands of star images on each plate. Stars don’t vary, so their brightness on each of the plates didn’t change. But scattered across this region of the sky were a handful of active galaxies that were slightly brighter on some plates and slightly fainter on others. Hunting down supermassive black holes is exciting, but comparing millions of images by staring at them with a simple eyepiece felt like a Sisyphean task.
Microlensing has some great advantages for exoplanet hunting and a few downsides. It’s sensitive to low mass planets and moons, and planets and moons orbiting at moderate to large distances from their stars. That makes it complementary to Doppler and transit searches, which work best at finding planets orbiting close to their stars. Microlensing can detect exoplanets at large distances, far across the Milky Way galaxy, and in principle even in other galaxies. If many thousands of stars are targeted and a microlensing event occurs in any of them, it will be detected.
The big downside is that the event never repeats. With Doppler and transit methods, the orbit repeats, data can be rechecked, and the results from the two methods can be combined. With microlensing, the two stars are widely separated in space and on different trajectories. Like ships passing in the night, they’ll never meet again.
Paczyński’s genius was realizing that small telescope networks could fill in a missing piece of astronomy: studying the behavior of variable objects, things that go bump in the night.
The method that Paczyński inspired has been a great success. Over a hundred exoplanets have been discovered in this way. Microlensing has found one of the lowest-mass exoplanets, just bigger than Earth’s mass, and one of the most massive, 13 times Jupiter’s mass. It’s the most sensitive method that astronomers possess for detecting dark objects. This latter object is so large that it straddles the boundary between planets and stars. It has even found one of the most robust candidates for an exomoon, making the fantasy of Pandora just a little more tangible.
Paczyński’s genius was realizing that small telescope networks could fill in a missing piece of astronomy: studying the behavior of variable objects, things that go bump in the night. “Most modern telescopes peer in great detail at tiny specks in the heavens,” he said. “They can’t keep track of what varies, pulsates or flares up and disappears forever.” He solved another variability puzzle: unexplained bursts of gamma rays discovered by satellites monitoring compliance with the Nuclear Test Ban Treaty. Almost alone, with most of his colleagues deeply skeptical, he argued that they originated far beyond the galaxy and were extremely luminous events. Paczyński was proved right in the 1990s by new satellite observations.
The father of microlensing was brilliant, but also plainspoken and modest. He worked tirelessly to support Polish astronomy after he emigrated, and almost single-handedly kept it going during the dark days of the Soviet regime. In 2003, he was diagnosed with brain cancer. Confined to a wheelchair, he continued to work and mentor young scientists. He faced his disease with courage and humor, but the cancer would not relent. His was a great mind passing in the night, shining brightly and then fading.
Chris Impey, a University Distinguished Professor of Astronomy at the University of Arizona, is the author of several books, including “Beyond: Our Future in Space,” “Einstein’s Monsters: The Life and Times of Black Holes,” and “Worlds Without End: Exoplanets, Habitability, and the Future of Humanity,” from which this article is adapted.